Converting Conventional Bacteria into Electrotrophs: A Novel Approach
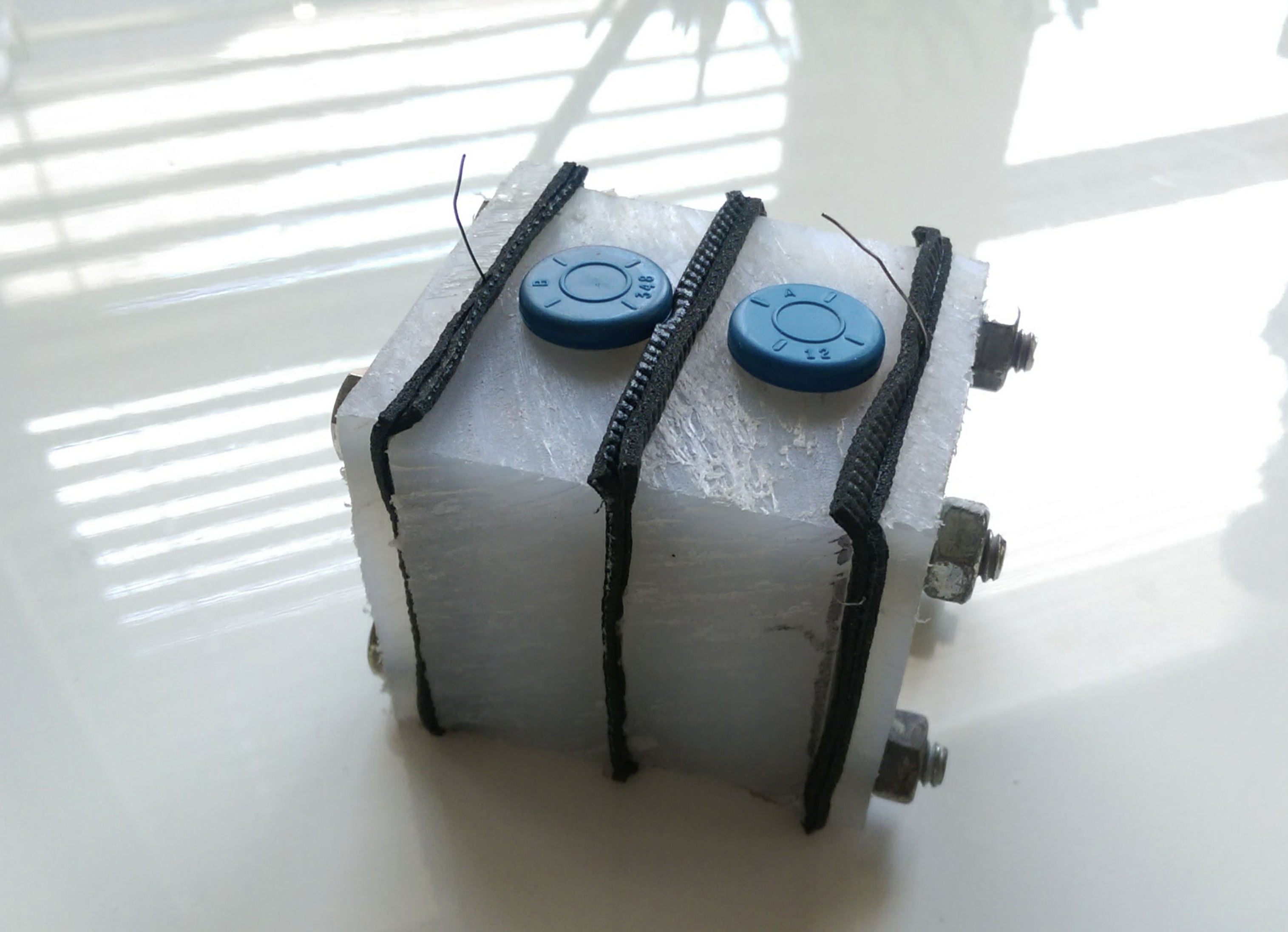
The ability of bacteria to carry out redox reactions for metabolic processes is well-documented. These reactions allow certain microorganisms to synthesize valuable byproducts like medicines, fuels, and plastics, or even extract toxic metals from water. However, these processes are often inefficient. This project focused on electrotrophs—bacteria capable of feeding on electrons directly from an electrode. Unlike conventional bacteria that rely on organic substrates, electrotrophs channel most of the electrons they receive into producing metabolic byproducts. This makes bioelectrochemical systems (BESs) more efficient, leading to higher yields of desired compounds and more effective environmental remediation. The goal of this project was to turn Escherichia coli K12, a non-electrotrophic strain, into an electrotroph and explore methods that could improve BES efficiency.
Project Background
Electrotrophs have significant potential for industrial applications. When used in BESs, they can synthesize hydrocarbons, plastics, and medicines with much greater efficiency than traditional bacterial processes. They can also be utilized for environmental remediation by efficiently precipitating toxic metals from water. However, the main limitation is that not all bacteria are electrotrophs, and those that are may not have desirable metabolic traits. Developing a method to convert conventional bacteria into electrotrophs is essential to advancing BES technologies and making them viable in large-scale applications.
Experimental Setup
To investigate this, I designed and built a BES system and ran three experimental groups under identical conditions. Each group consisted of three BESs:
- A sterile control BES.
- A BES inoculated with wild-type E. coli K12.
- A BES inoculated with a mutant strain of E. coli created through UV mutagenesis.
The objective was to enrich and isolate electrotrophic strains over multiple generations. Current outputs were recorded and analyzed for all groups, and pure electrotroph strains were isolated for further study.
BES Construction and Operation
The BES was constructed using a two-chamber design with carbon cloth electrodes, titanium wire, proton exchange membranes, and polypropylene sheets. Potassium ferricyanide and phosphate-buffered saline (PBS) were used as the catholyte for bioanode runs, while potassium ferrocyanide and PBS were used as the anolyte for biocathode runs.
The BESs were operated for 16-hour cycles at 37°C over seven generations. Each cycle included the extraction of 50% of the electrolyte, followed by sampling from the “electro-active” bioelectrode. The sample was grown in Luria broth (LB) and used to inoculate the next generation of the BES. Nitrogen was periodically bubbled through the chambers to maintain anaerobic conditions.
Biofilm Enrichment and Electrotroph Selection
Building stable biocathodes proved to be a significant challenge, as biocathode development is typically more difficult and time-consuming than bioanode formation. To expedite the process, I first grew bioanodes using potassium ferricyanide as the electron acceptor. After three generations, the BES was reversed using potassium ferrocyanide as the electron donor, turning the bioanodes into biocathodes and initiating the electrotroph selection process. Samples from the biocathode were streak-plated, and nine colonies were selected and grown in BESs to evaluate pure culture power outputs.
Results and Analysis
Power output was calculated using the microbial fuel cell power density equation:
Pcat = (Ecell × I) / Acat
Where:
- Pcat is the power density.
- Ecell is the cell voltage.
- I is the current.
- Acat is the area of the cathode.
Both the wild-type and mutant strain BESs showed improved electron transport efficiency compared to the control, with the mutant strain outperforming the wild-type in both bioanode and biocathode phases. After reversing the polarity, the mutant strain immediately demonstrated higher power output, suggesting successful selection of electrotrophic traits.
Pure strain analysis revealed that the highest power output came from Sample 3, though it was lower than that of the mixed culture BES. This was expected, as mixed cultures typically achieve higher power densities due to synergistic interactions between different bacterial strains.
Real-World Applications and Conclusion
This process demonstrates a novel method for converting conventional bacteria into electrotrophs, offering enhanced metabolic efficiency for applications in industrial production and environmental remediation. The approach also provides a more reliable way to isolate electrotrophic strains, addressing one of the major challenges in BES development.
The findings from this study represent a step forward in optimizing BESs, paving the way for future innovations in sustainable chemical production and resource recovery.